Stars are the most basic building blocks of galaxies.
The age, distribution, and composition of stars trace the history, dynamics, and evolution of their galaxy. Stars are responsible for the production and distribution of heavy elements, such as carbon, nitrogen, and oxygen.
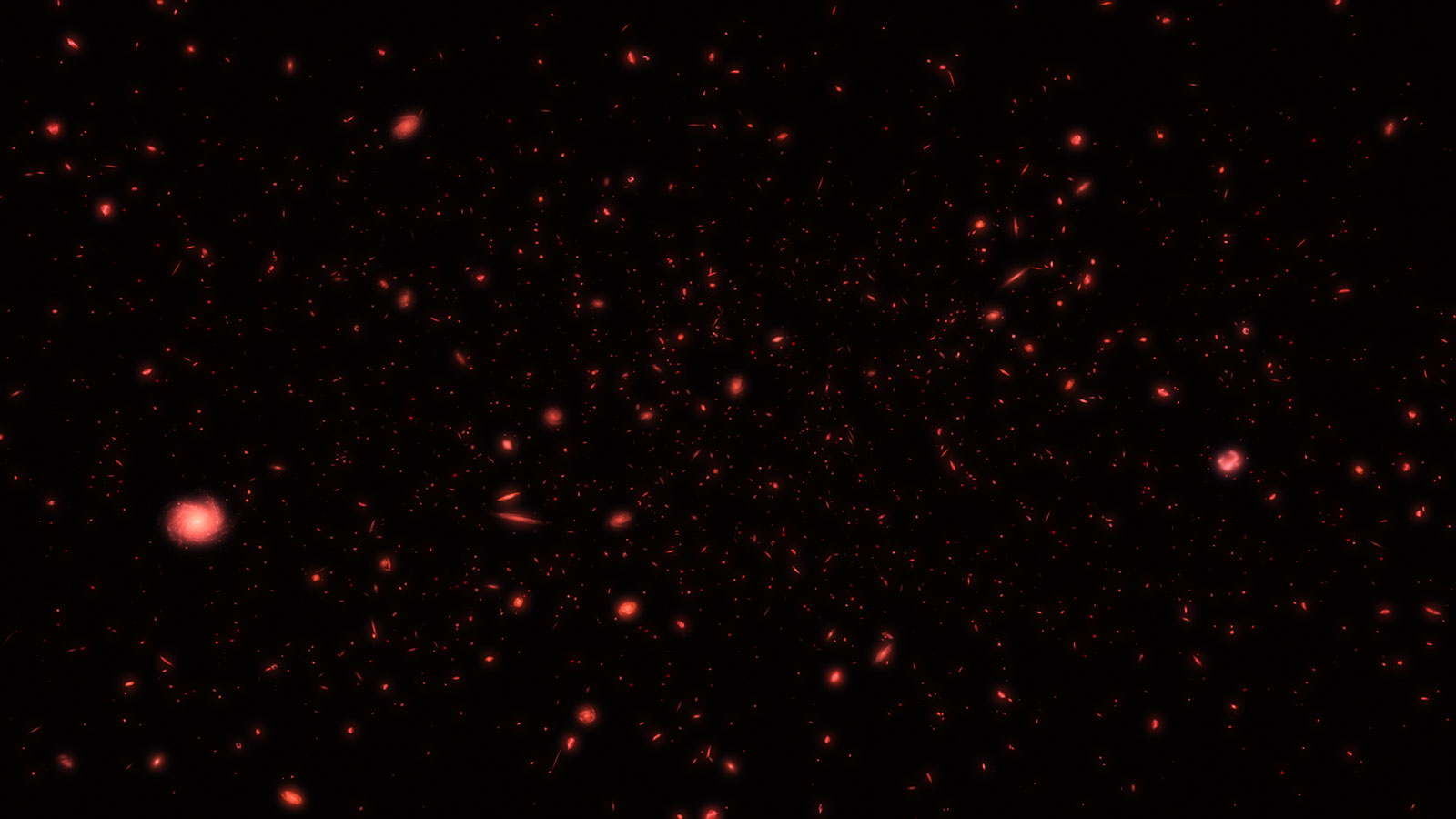
Different star types have different habitable zones. This is the area around a star where conditions are just right, neither too hot nor too cold for liquid water to exist on a planet’s surface. (For this reason, a star's habitable zone is often referred to informally as its "Goldilocks zone.")
Statistically, there should be more than 100 billion planets in our Milky Way galaxy. They come in a wide range of sizes and characteristics. Complex organisms arose on Earth only 500 million years ago, and modern humans have been here for only 200,000 years – the blink of an eye on cosmological timescales. Earth will become uninhabitable for higher forms of life in a little over 1 billion years, as the Sun grows warmer and dries our planet. Therefore, stars slightly cooler than our Sun – called orange dwarfs – are considered better for advanced life. They can burn steadily for tens of billions of years. This opens up a vast timescape for biological evolution to pursue an infinity of experiments for yielding robust life forms. And, for every star like our Sun, there are three times as many orange dwarfs in the Milky Way.
The even more abundant star type called red dwarfs (also known as M dwarf stars) have even longer lifetimes. Planets in a red dwarf's comparatively narrow habitable zone, which is very close to the star, are exposed to extreme levels of X-ray and ultraviolet radiation, which can be hundreds of thousands of times more intense than what Earth receives from the Sun. Planets in the habitable zones of red dwarfs can be baked bone dry and have their atmospheres stripped away quite early in their lives. Red dwarfs typically calm down after a few billion years, but their early outbursts could prohibit their planets from evolving to be more hospitable.
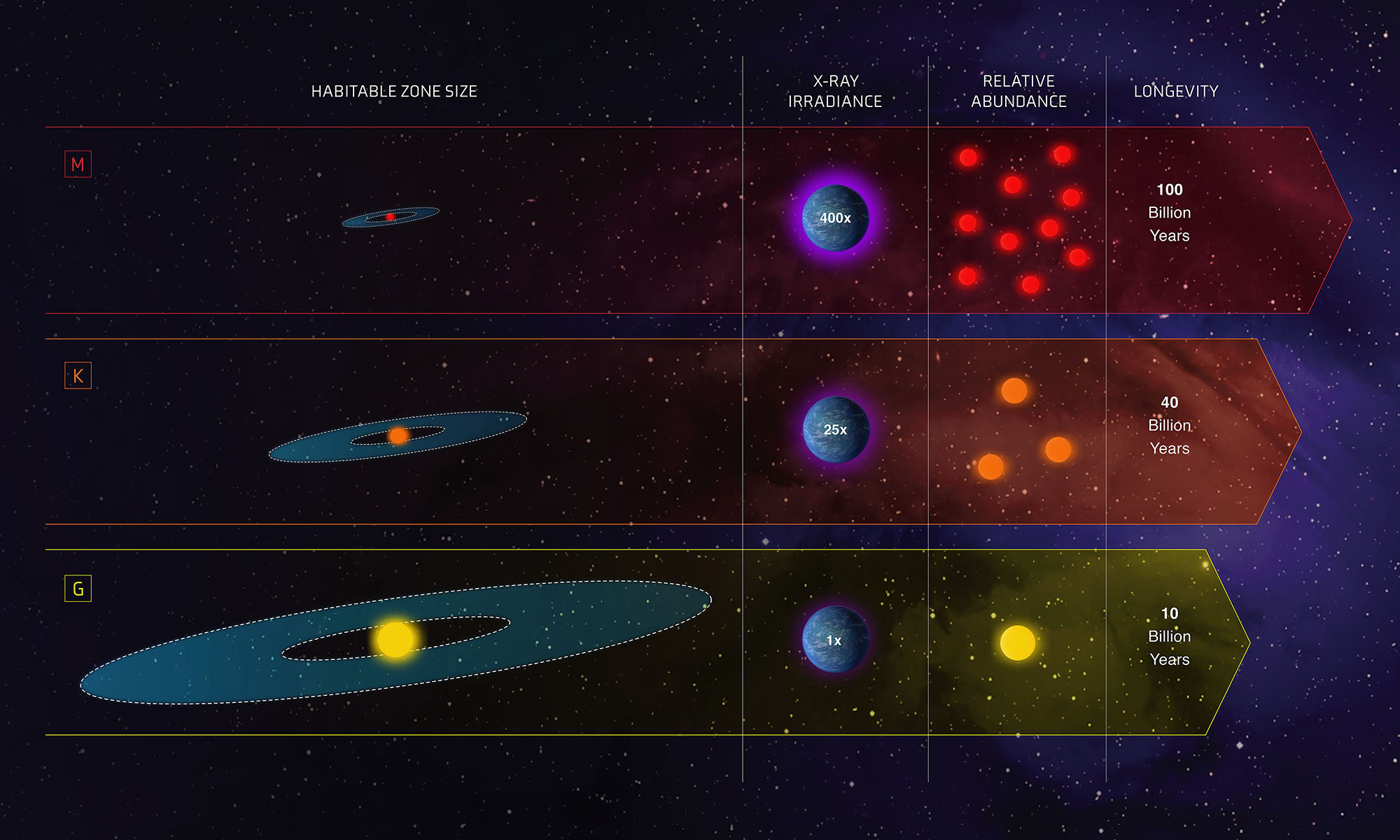
Stars are born from vast clouds of gas and dust, known as nebulae, that are scattered throughout most galaxies. Over thousands to millions of years, gravity can cause denser pockets within a nebula to collapse under their own weight. As a cloud – which is mostly hydrogen – collapses, the material at its center begins to heat up. Known as a “protostar,” this hot core of the collapsing cloud will is a star in the making. Some of these spinning clouds of collapsing gas and dust break up into two or three blobs that each form stars. This would explain why most of the stars in the Milky Way come in pairs or in multiples. Not all of this material ends up as part of the star, however – the remaining dust can become planets and moons, asteroids, and comets – or may simply remain as dust.
As millions of years pass, the core temperature of a protostar reaches a point at which nuclear fusion can begin. The star then begins the longest stage of its life, called the “main sequence.” Most stars in the galaxy, including our Sun, are categorized as main sequence. This is a state in which nuclear fusion in the star is stable and hydrogen is converted to helium. This process releases a lot of energy that keeps the star hot and bright, and it supplies an outward pressure against the incredible mass of material that would otherwise cause the star to collapse on itself. Ninety percent of a star’s life is spent in the main sequence phase.
When you look at the night sky, you may notice that some stars shine more brightly than others. The brightness of a star is related to how much energy it puts out, as well as how close it is to Earth.
Stars also vary in color – because they vary in temperature. Hotter stars appear blue or white, while cooler stars look orange or red. Astronomers use these characteristics to classify main sequence stars into categories by color and temperature: O (blue), B (blue-white), A (white), F (yellow-white), G (yellow), K (orange), and M (red), from hottest and biggest to coolest and smallest. Stars at the ends of their lives are out of the main sequence. These include supergiants, red giants, and white dwarfs.
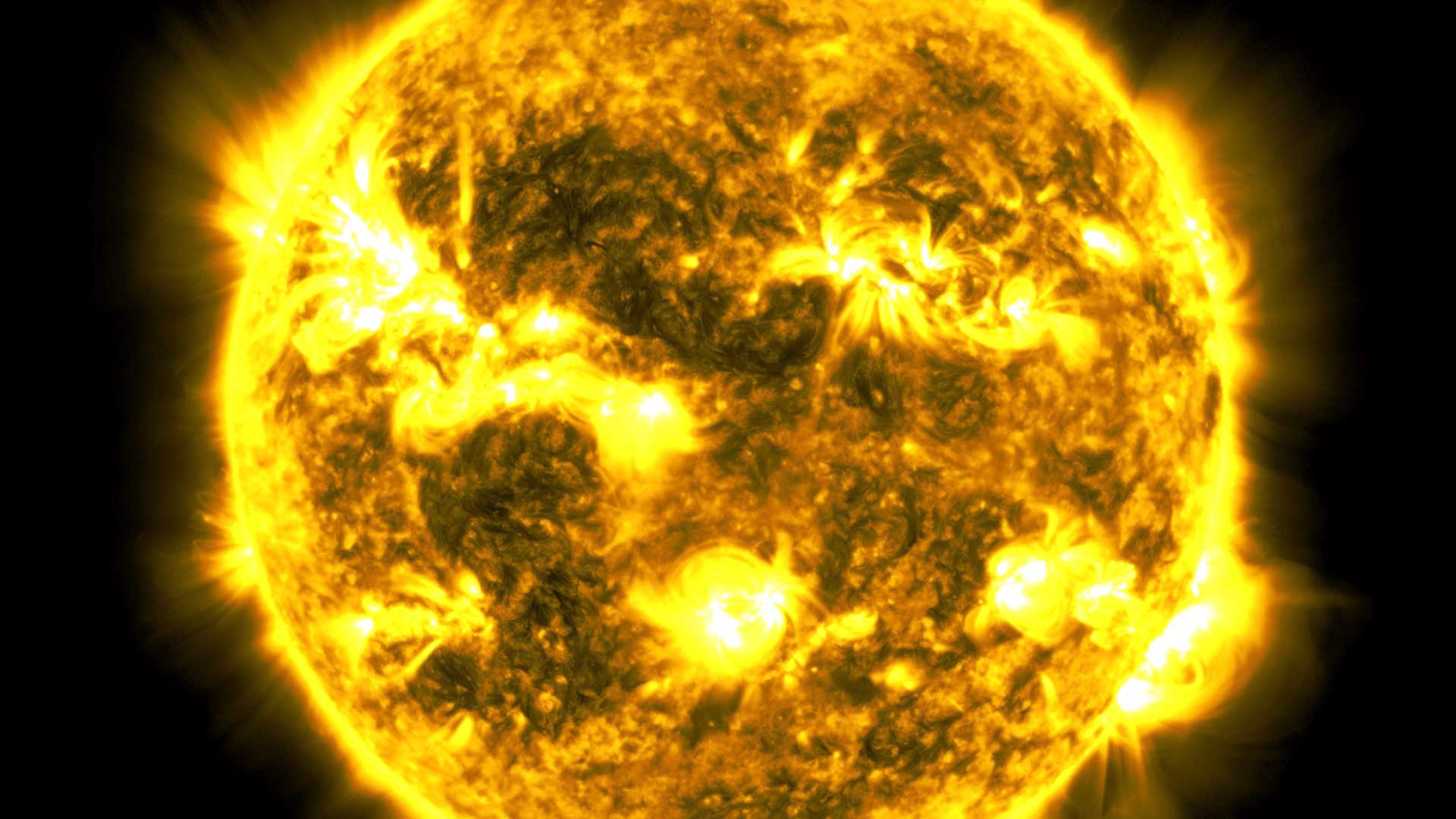
Our Sun is categorized as a G-type yellow-dwarf main sequence star. It is predicted that our Sun will remain in the main sequence phase for a few billion more years.
Stars can live for billions of years, but their lives can be shorter or longer depending on their size (technically, their mass). The bigger (or more massive) the star, the shorter its life, as more massive stars burn their nuclear fuel faster.
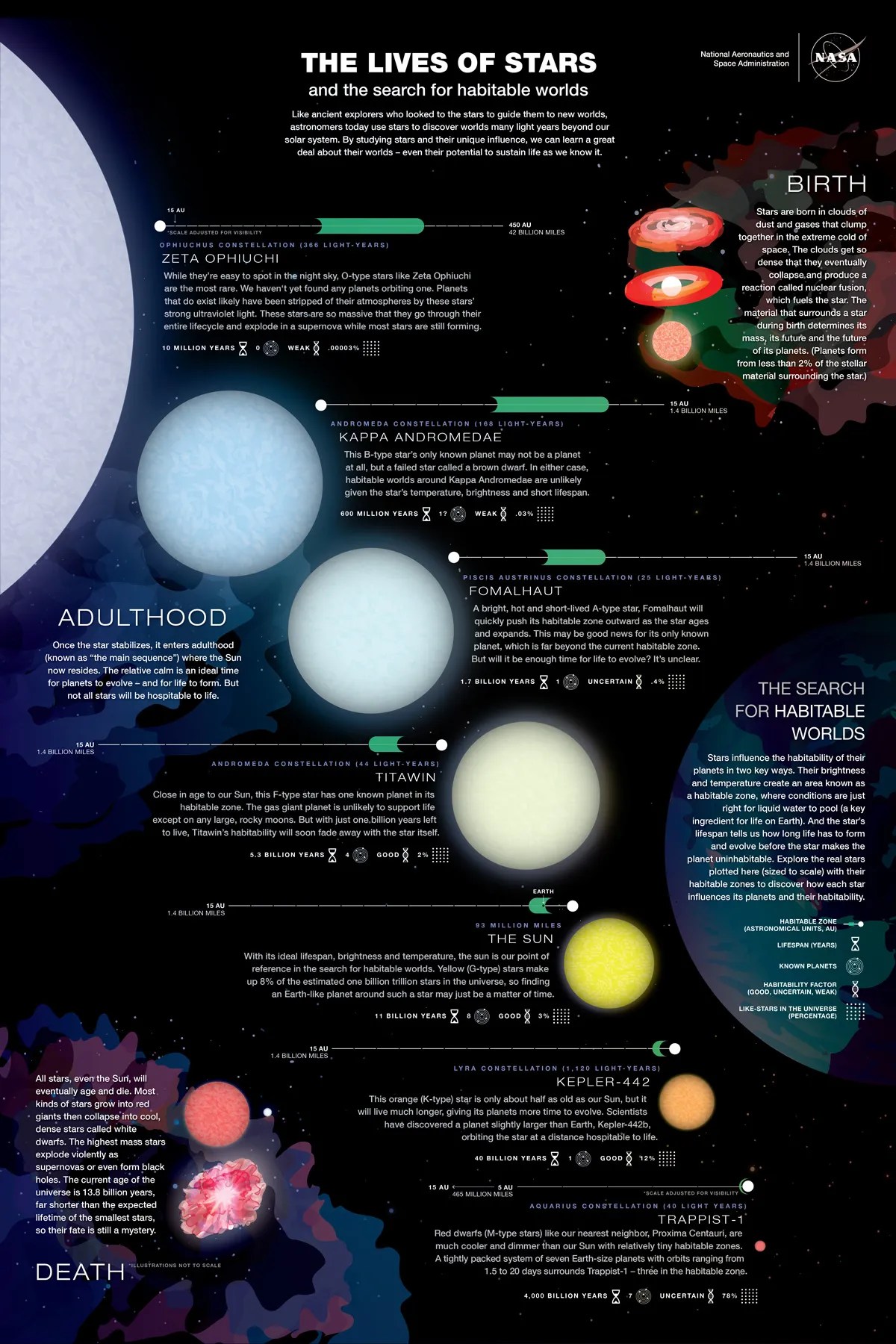
The gas and dust that swirl around a star during its formation are critical to forming planets around it. The dust contains heavy elements such as carbon and iron that form the cores of planets.
Scientists think planets start off as grains of dust smaller than the width of a human hair. They emerge from the giant, donut-shaped disk of gas and dust that circles young stars. Gravity and other forces cause material within the disk to collide. If the collision is gentle enough, the material fuses, growing like rolling snowballs. Over time, dust particles combine to form pebbles, which evolve into mile-sized rocks. As these planetesimals, or planets in the making, orbit their star, they clear material from their path, leaving tracks of space empty but for fine dust. At the same time, the star gobbles up nearby gas while pushing more distant material farther away. After a few million years, the disk will have totally transformed, much of it taking the form of new worlds.
When an average star like our Sun runs out of hydrogen to fuse, the star starts to collapse. But compacting a star causes it to heat up again and it is able to fuse what little hydrogen remains in a shell wrapped around its core. This burning shell of hydrogen greatly expands the outer layers of the star. When this happens, the star becomes a red giant. When our Sun enters the red giant phase of its life, in about 5 billion years, it will be so big that Mercury will be completely swallowed.
Our red giant Sun will still be consuming helium and cranking out carbon. When the helium is gone, the Sun will succumb to gravity again. When the core contracts, it will cause a release of energy and the Sun will become an even bigger giant with a radius beyond Earth's orbit.
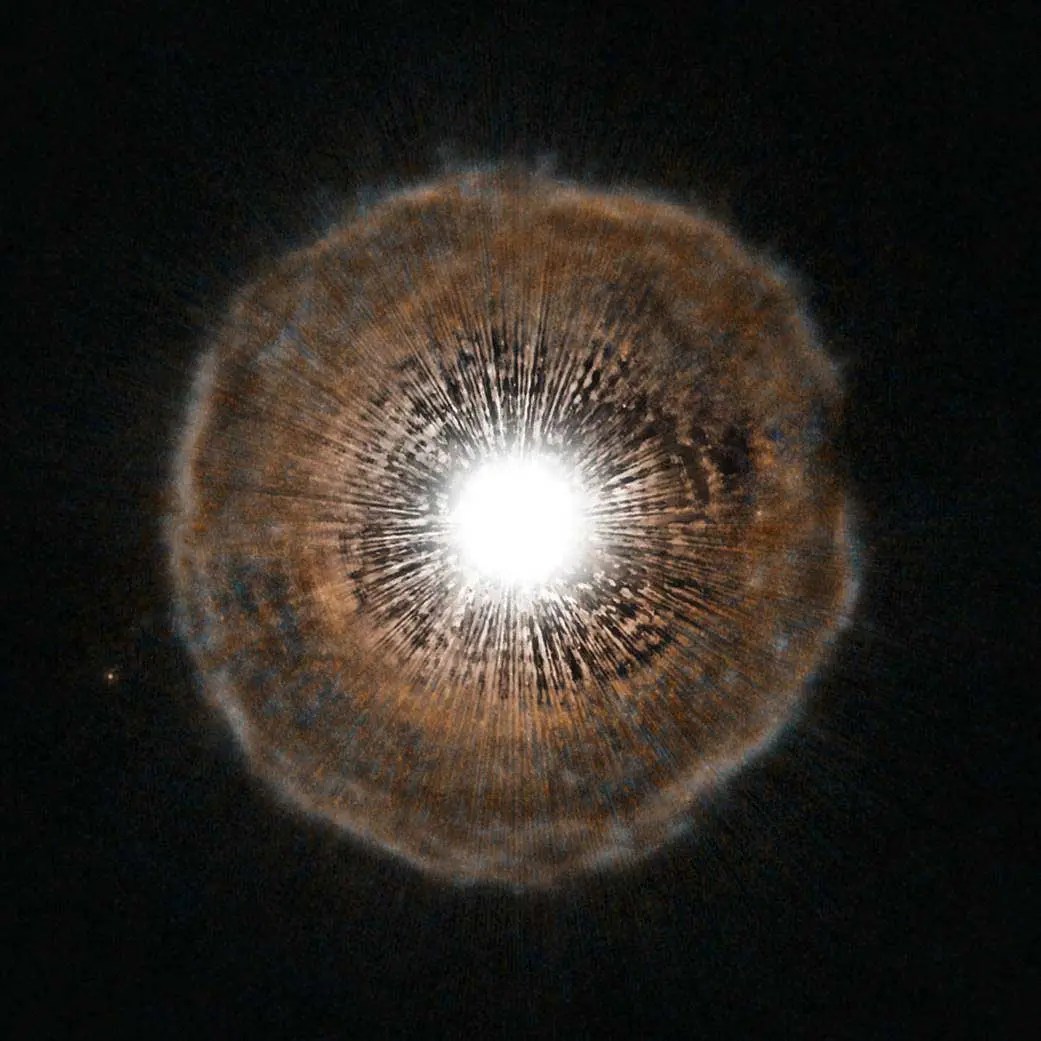
After about a billion years as a red giant, the Sun will have ejected its outer layers until, eventually, its stellar core is exposed. This dead (in terms of nuclear fusion) but still ferociously hot stellar cinder is called a white dwarf. White dwarfs are roughly the size of Earth, despite containing the mass of a star. Pressure from fast moving electrons keeps these stars from further collapse. The more massive the core, the denser the white dwarf that is formed. Thus, the smaller a white dwarf is in diameter, the larger it is in mass! White dwarfs fade into oblivion over many billions of years as they gradually cool down.
This fate awaits only those stars with a mass up to about 1.4 times the mass of our Sun. Above that mass, electron pressure cannot support the core against further collapse. Such stars suffer a different fate.
If a white dwarf forms in a binary or multiple star system, it may experience a more eventful demise as a nova. Nova is Latin for "new" – novae were once thought to be new stars in the act of being born. Today, we understand that they are very old stars – white dwarfs. If a white dwarf is close enough to a companion star, its gravity may drag matter (mostly hydrogen) from the outer layers of that star onto itself, building up on its surface. When enough hydrogen has accumulated on the surface, a burst of nuclear fusion erupts, causing the white dwarf to brighten substantially and eject its remaining material. Within a few days, the glow subsides and the cycle starts again. Sometimes, particularly massive white dwarfs (those near the 1.4 solar mass limit) may accrete so much mass in this manner that they collapse and explode completely, becoming what is known as a supernova.
Stars more than eight times the mass of our Sun are destined to die in a titanic explosion called a supernova. A supernova is not merely a bigger nova. In a nova, only the star's surface explodes. In a supernova, the star's core collapses and then explodes. In massive stars, a complex series of nuclear reactions leads to the production of iron in the core. Having achieved iron, the star has wrung all the energy it can out of nuclear fusion. The star no longer has any way to support its own mass, and the iron core collapses. In just a matter of seconds the core shrinks from roughly 5,000 miles across to just a dozen, and the temperature spikes 100 billion degrees or more. The outer layers of the star initially begin to collapse along with the core, but rebound with the enormous release of energy and are thrown violently outward. Supernovae release an almost unimaginable amount of energy. For a period of days to weeks, a supernova may outshine an entire galaxy. Likewise, all the naturally occurring elements and a rich array of subatomic particles are produced in these explosions.
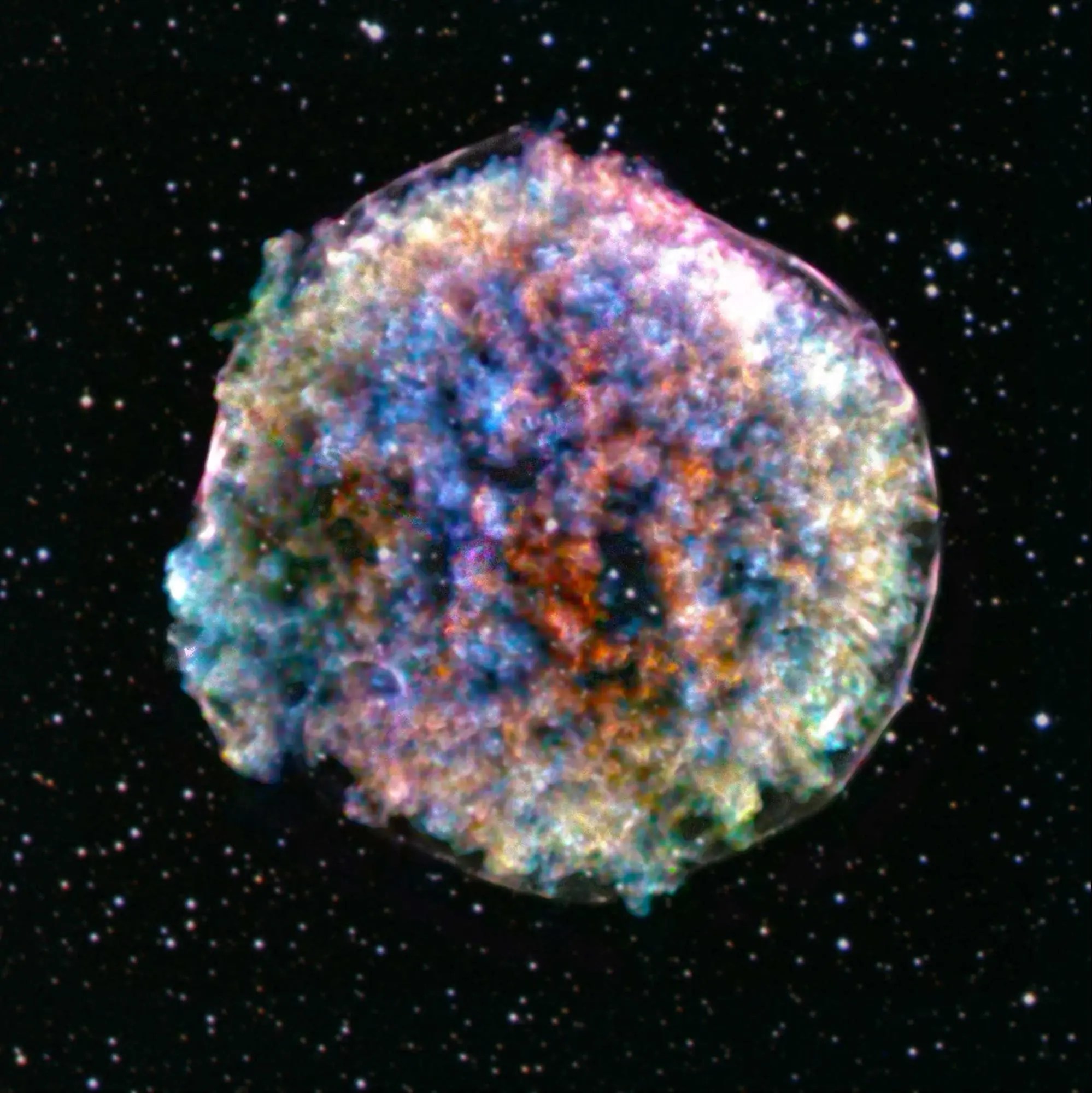
If the collapsing stellar core at the center of a supernova contains between about 1.4 and 3 solar masses, the collapse continues until electrons and protons combine to form neutrons, producing a neutron star. Neutron stars are incredibly dense. Because it contains so much mass packed into such a small volume, the gravity at the surface of a neutron star is immense. Like white dwarfs, if a neutron star forms in a multiple star system it can accrete gas by stripping it from nearby companions.
Neutron stars also have powerful magnetic fields that can accelerate atomic particles around its magnetic poles, producing powerful beams of radiation. Those beams sweep around like massive searchlights as the star rotates. If such a beam is oriented so that it periodically points toward Earth, we observe it as regular pulses of radiation that occur whenever the magnetic pole sweeps past our line of sight. In this case, the neutron star is known as a pulsar.
If the collapsed stellar core is larger than three solar masses, it collapses completely to form a black hole: an infinitely dense object whose gravity is so strong that nothing can escape, not even light.
Because photons are what our instruments are designed to see, black holes can only be detected indirectly. Indirect observations are possible because the gravitational field of a black hole is so powerful that any nearby material – often the outer layers of a companion star – is caught up and dragged in. As matter spirals into a black hole, it forms a disk, called an accretion disk, that is heated to enormous temperatures, emitting copious quantities of X-rays and gamma-rays that indicate the presence of the underlying hidden companion.
Black holes that are quiet and not actively "feeding" on accretion disks can also be detected indirectly by observing the motions of nearby stars. For example, astronomers observe the supermassive black hole at the center of the Milky Way by watching as nearby stars whip around at astounding speeds only possible under the influence of an incredibly massive, but invisible object.
The dust and debris left behind by novae and supernovae, as well as by red giants puffing off their outer layers, eventually blend with the surrounding interstellar gas and dust, forming new nebulae. The products created in the ends of the lives of stars enrich galaxies with heavy elements and chemical compounds. Eventually, those materials are recycled, providing the building blocks for new generations of stars and planetary systems.