Energy Dissipation in Collisionless Shocks
January 19, 2015
Energy Dissipation in Collisionless Shocks, by Lynn B Wilson
In the 1960s, scientists discovered a new kind of shock wave that traveled through space plasmas that did not rely upon collisions. Thus, they are known as collisionless shock waves. These shocks are of great interest in multiple fields of research: they can produce radiation that can negatively impact commercial and military spacecraft operation, as well as the safety of humans in space.
But the mechanisms allowing these shocks to form has been a topic of great debate. Our recent work helps resolve some of these issues by confirming theories that predict how small-scale phenomena can control large-scale dynamics. These small-scale processes, on the order of tens of meters, can regulate structures at scales more than 1 million meters across.
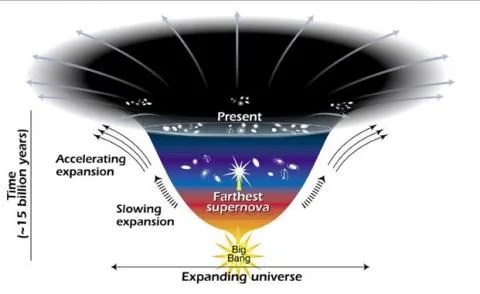
Common shock waves – such as those at the front of a supersonic jet -- occur when an obstacle moves faster than the speed of sound, that is, the speed of a compression wave through a fluid. Such shocks transform the bulk flow from supersonic to subsonic in a thin transition region called the shock ramp, where the lost kinetic energy is converted into heat. With common shock waves, this conversion occurs mostly through binary particle collisions, much like billiard balls colliding and recoiling. These collisions occur over a short distance called the particle mean free path, which, in Earth's atmosphere, is around a micrometer.
In the magnetized bow shock in front of Earth, however, the particle mean free path in the solar wind can be as large as one astronomical unit, that is, the distance between the Earth and the sun or around 150 billion meters. On the other hand, the magnetized shock ramps were found to be less than one-millionth that size. There was no way these shocks could rely upon particle collisions taking place over billions of meters -- thus the name collisionless shock wave. But how could such shocks transform the incident bulk flow into heat in such short distances without collisions?
Four primary mechanisms were proposed. Three of the theories, however, were proposed as steps that, in fact, created a fourth process named anomalous resistivity. Our study focuses on the fourth mechanism, which occurs so quickly that it could only recently be tested using the high cadence field and particle measurements of the THEMIS spacecraft.
The theory for the fourth mechanism arose by recognizing that electromagnetic waves could impede the relative velocities between electrons and ions (i.e., electric currents) that give rise to magnetic shock ramps. These waves can transfer momentum to charged particles by scattering them off their original trajectories and/or acting as an effective drag force, slowing the bulk flows that produce electric currents. This extra resistance gave rise to the name anomalous resistivity. More recently, however, the more generic term wave-particle interactions has been used, as not all of these interactions affect the electric currents.
Since wave-particle interactions occur much faster than particle instruments can currently measure, testing their relative importance poses a problem. We found a way around this issue by estimating the electric currents in collisionless shock ramps by using a combination of magnetic field measurements and the laws governing electromagnetism --Maxwell’s equations. We could then estimate the energy dissipation rate due to wave-particle interactions. The final step in the analysis involved estimating the total energy dissipation rate needed to maintain a stable shock on large-scales.
The results were surprising. First, we discovered that not only are small-scale waves ubiquitous in collisionless shock waves -- but they can be huge. In some cases, the wave amplitudes were so large that they contained as much energy density as is necessary to produce an aurora. Second, we found that the energy dissipation rates due to wave-particle interactions were also very large. So large, in fact, that they could exceed the large-scale dissipation rates by over 10,000 times. In other words, the wave-particle interactions need only be ~0.01% efficient and they could still regulate the large-scale structure of the shock.
This information about waves near Earth can also be extrapolated to inaccessible regions of space. We found that similar processes could provide enough energy to explain the heating of the solar corona, magnetic reconnection rates, and have implications for particle heating and acceleration around stars elsewhere in the universe. These results quantitatively show, for the first time, that small-scale phenomena can control the large-scale dynamics in collisionless plasmas.
Citation: Wilson et al., in the Journal of Geophysical Research Vol. 119, with titles “Quantified energy dissipation rates in the terrestrial bow shock: 1. Analysis techniques and methodology” and “Quantified energy dissipation rates in the terrestrial bow shock: 2. Waves and dissipation.”