Chapter 16: Encounter
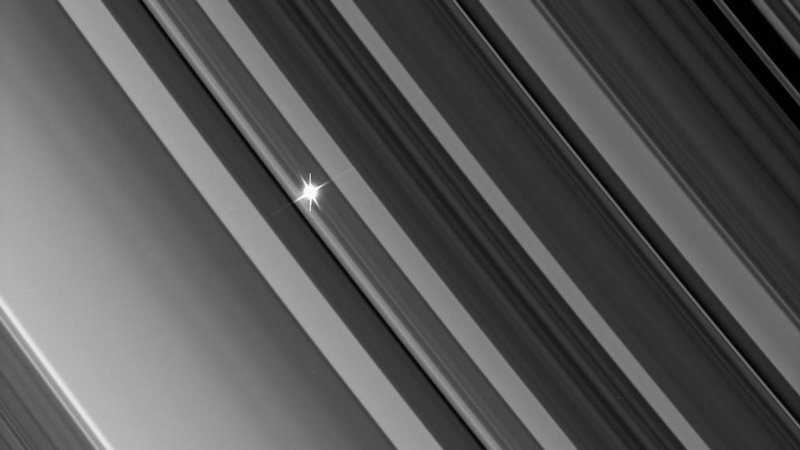
Occultations provide unique opportunities to conduct scientific experiments. Occultations of interest include the earth, the Sun, or another star disappearing behind a planet, behind its rings, or behind its atmosphere, as viewed from the spacecraft. They are of interest when involving either the main planet or its satellites. During the one-time only opportunity for occultation by a planet during a flyby mission encounter, or repeatedly during an orbital mission, onboard instruments may make unique observations. Here are a few examples:
- An ultraviolet spectrometer watches the Sun as it disappears behind a planet's atmosphere (or a satellite's atmosphere), obtaining spectra deep into the atmosphere, that can be studied to determine its composition and structure.
- A photometer watches a bright distant star as it passes behind a ring system. Results yield high-resolution data on the sizes and structures of the ring system and its particles.
- The telecommunications system is used by Radio Science to measure the Doppler shift around closest approach to a satellite, to determine the object's mass.
- Radar Science commandeers the telecommunications system to perform a bistatic radar experiment: The Deep Space Network (DSN) observes the radio signal glancing off a satellite's surface as the spacecraft directs its downlink signal at just the right spots on the satellite to achieve this specular reflection. Results can yield information on the structure and composition of the surface. (While this kind of experiment doesn't actually need an occultation, it might be performed near occultation.)
- Radio Science observes the spacecraft's radio signal on Earth as the spacecraft passes behind a planet or a satellite, yielding data on the composition and structure of its atmosphere (see Chapter 8).
- Radio Science ring occultations provide data on the ring system's structure and composition. Of course this involves recording the downlink as the DSN sees it through the rings. The spacecraft's transmission is trained on Earth as the rings drift by.
Recall that the most precise Radio Science investigations require a two- or three-way coherent mode, receiving an uplink from the DSN as discussed in Chapter 10. However, with an atmospheric occultation, or an occultation of an opaque ring section, this coherent link is generally possible on ingress only; the spacecraft is likely to lose the uplink from DSN when it passes close behind the planet, and therefore would be incapable of producing a coherent downlink. For this reason, some spacecraft are equipped with an Ultra Stable Oscillator (USO) in a temperature-controlled "oven" on the spacecraft which is capable of providing a fairly stable downlink frequency for a short time when an uplink is not available. Calibrations, of course would, be accomplished shortly before and after the occultation.
The first occultation experiment was proposed when JPL was characterizing the precise refraction effects of Earth's atmosphere, with its known structure and composition, for the purpose of tracking spacecraft. The experimenter realized that measurements of the refraction effects induced by another planet's atmosphere could be used to "reverse-engineer" its structure and composition!
Occultation may be one method of identifying extrasolar planets (also known as "exoplanets") by measuring the slightly reduced brightness of a distant star as one of its planets happens to pass in front of it as viewed from Earth. A spacecraft is not necessarily required for this kind of measurement; it can be undertaken to some extent using earth-based telescopes.
Somewhat related to this subject, many Earth-based telescopes, and some proposed orbiting instruments, are equipped with an occulting disk coronograph) that can block out light from the central star. This facilitates examining the star's "planetary" disk of material orbiting it. Scattered light in the instrument and/or in the Earth's atmosphere limits its effectiveness, but studies exist for orbiting an external large occulting disk to improve experiments' effectiveness from Earth-based or orbiting telescopes.
Planets are not perfectly spherical. Terrestrial planets are rough-surfaced, and most planets are at least slightly oblate. Thus they have variations in their mass concentrations, sometimes associated with mountain ranges or other features visible on the surface. A gravity field survey, as introduced in Chapter 8, identifies local areas of a planet that exhibit slightly more or slightly less gravitational attraction. These differences are due to the variation of mass distribution on and beneath the surface.
There are two reasons for surveying the gravity field of a planet. First, highly accurate navigation in orbit at a planet requires a good model of variations in the gravity field, which can be obtained by such a survey. Second, gravity field measurements have the unique advantage of offering scientists a "view" of mass distribution both at and below the surface. They are extremely valuable in determining the nature and origin of features identifiable in imaging data. JPL has pioneered the field of mapping planetary mass concentrations. Application of these techniques to Earth helps geologists locate petroleum and mineral deposits, as well as provide insight to geological processes at work.
To obtain gravity field data, a spacecraft is required to provide only a downlink carrier signal coherent with a highly stable uplink from the DSN. It may be modulated with telemetry, command and ranging data, or it may be unmodulated. After the removal of known Doppler shifts induced by planetary motions and the spacecraft's primary orbit, and other factors, the residual Doppler shifts are indicative of miniscule spacecraft accelerations resulting from variations in mass distribution at, and below, the surface of the planet. The gravity feature size that can be resolved is roughly equal to the spacecraft's altitude; with a 250-km altitude, a spacecraft should resolve gravity features down to roughly 250 km in diameter.
With an X-band (3.6 cm wavelength) uplink received at a spacecraft, and a coherent X-band downlink, spacecraft accelerations can be measured to tens of micrometers per second squared. This translates to a sensitivity of milligals in a planetary gravity field. (One gal represents a gravitational acceleration of 1 cm/sec2).
The most accurate and complete gravity field coverage is obtained from low circular orbit. Mars Global Surveyor conducted gravity field surveys from circular orbit as one of its first-priority investigations. Magellan's orbit was elliptical during its primary mission, and meaningful gravity data could be taken only for the portion of the orbit that was plus and minus about 30 degrees true anomaly from periapsis, which occurred at about 10 degrees north latitude. After aerobraking (see below) to a low circular orbit, Magellan conducted a high-resolution gravity field survey of the entire planet.

Aerobraking is the process of decelerating by converting velocity mostly into heat via supersonic compression in a planetary atmosphere.
Galileo's atmospheric probe is a typical example of an atmospheric entry and aerobraking mission. The probe was designed with an aeroshell that sustained thousands of degrees of heat as it entered the Jovian atmosphere. In fact its aeroshell reached a higher temperature than the Sun's photosphere. It decelerated at hundreds of Gs, until it reached a speed where its parachute became effective.
At that time, the spent aeroshell was discarded, and the probe successfully carried out its experiments characterizing Jupiter's upper atmosphere.

The Magellan spacecraft was not designed for atmospheric entry. However, the periapsis altitude of Magellan's orbit was lowered, by the use of propulsive maneuvers, into the upper reaches of Venus's atmosphere near 140 km above the surface. This is still high above the cloudtops, which are at about 70 km.
Flying at this altitude induced deceleration via atmospheric friction during the portion of the spacecraft's orbit near periapsis, thus reducing the height to which it could climb to apoapsis (recall the discussions in Chapter 3.
The solar array, consisting of two large square panels, was kept flat-on to the velocity vector during each pass through the atmosphere, while the HGA trailed in the wind. The solar array reached a maximum of 160° C, and the HGA a maximum of 180° C.
After approximately 70 earth days and one thousand orbits of encountering the free molecular flow, and decelerating a total of about 1250 m/sec, the apoapsis altitude was lowered to a desirable altitude. The periapsis altitude was then raised to achieve a nearly circular orbit.
The objectives of this aerobraking experiment were to demonstrate the use of aerobraking for use on future missions, to characterize the upper atmosphere of Venus, and to be in position to conduct a full-planet gravity field survey from a nearly circular orbit.

Landing on a planet is generally accomplished first by aerobraking while entering the planet's atmosphere under the protection of an aeroshell. From there, the lander might be designed to parachute to the surface, or to use a propulsion system to soft-land, or both, as did the Viking landers on Mars. In addition to aeroshell, parachutes and propulsion, the Mars Pathfinder spacecraft used airbags to cushion its impact. This technique was repeated with the Mars Exploration Rovers, Spirit and Opportunity.
After aerobraking and parachuting, the Mars Science Laboratory rover named Curiosity was lowered to the surface using a propulsive descent module known as a Sky Crane.
The Soviet Venera spacecraft parachuted to the surface of Venus by means of a small rigid disk integral with the spacecrafts' structure which helped slow their descent sufficiently through the very dense atmosphere. A crushable foot pad absorbed the energy from their final impact on the surface.
Even though Huygens enjoyed the immense fortune of surviving impact with the surface of Titan, and returning data for over 90 minutes, the mission was classified as an atmospheric probe, not a lander. Its prime mission was to collect data while descending for more than two hours through the atmosphere.
JPL's Surveyor missions landed on the Moon via propulsive descent, coming to rest on crushable foot pads at the lunar surface.
For a possible future mission, some members of the international science community desire to land a network of seismometer-equipped spacecraft on the surface of Venus to measure seismic activity over a period of months or years.

Once deployed within a planet's atmosphere, having undergone atmospheric entry operations as discussed above, a balloon may ride with the wind and depend on the DSN to directly track its progress, or it can use an orbiting spacecraft to relay its data to Earth. In 1986, DSN tracked the Venus balloons deployed by the Soviet Vega spacecraft when it was on its way to encounter comet Halley. The process of tracking the balloon across the disc of Venus yielded data on the circulation of the planet's atmosphere.
The planned Mars Balloon is designed to descend to just above the surface. Carrying an instrument package, including a camera, within a long, snake- or rope-like structure, it will rise and float when heated by the daytime Sunlight, and will sink and allow the "rope" to rest on the surface at night. In this way it is hoped that the balloon package will visit many different locations pseudo-randomly as the winds carry it. In doing so, it will also yield information on atmospheric circulation patterns. The Mars balloon is designed jointly by Russia, CNES, and The Planetary Society, a public non-profit space-interest group in Pasadena. It will depend upon an orbiting spacecraft to relay its data home.
The Mars Global Surveyor spacecraft carried radio relay equipment (see Chapter 11) designed to relay information from landers, surface penetrators, balloons, or Mars aircraft. The Mars Odyssey and Mars Express orbiters are similarly equipped. Future Mars orbiting spacecraft will also have relay capability, as did two Mars-bound spacecraft that were lost: Mars Observer in 1993 and Mars Climate Orbiter in 1999.
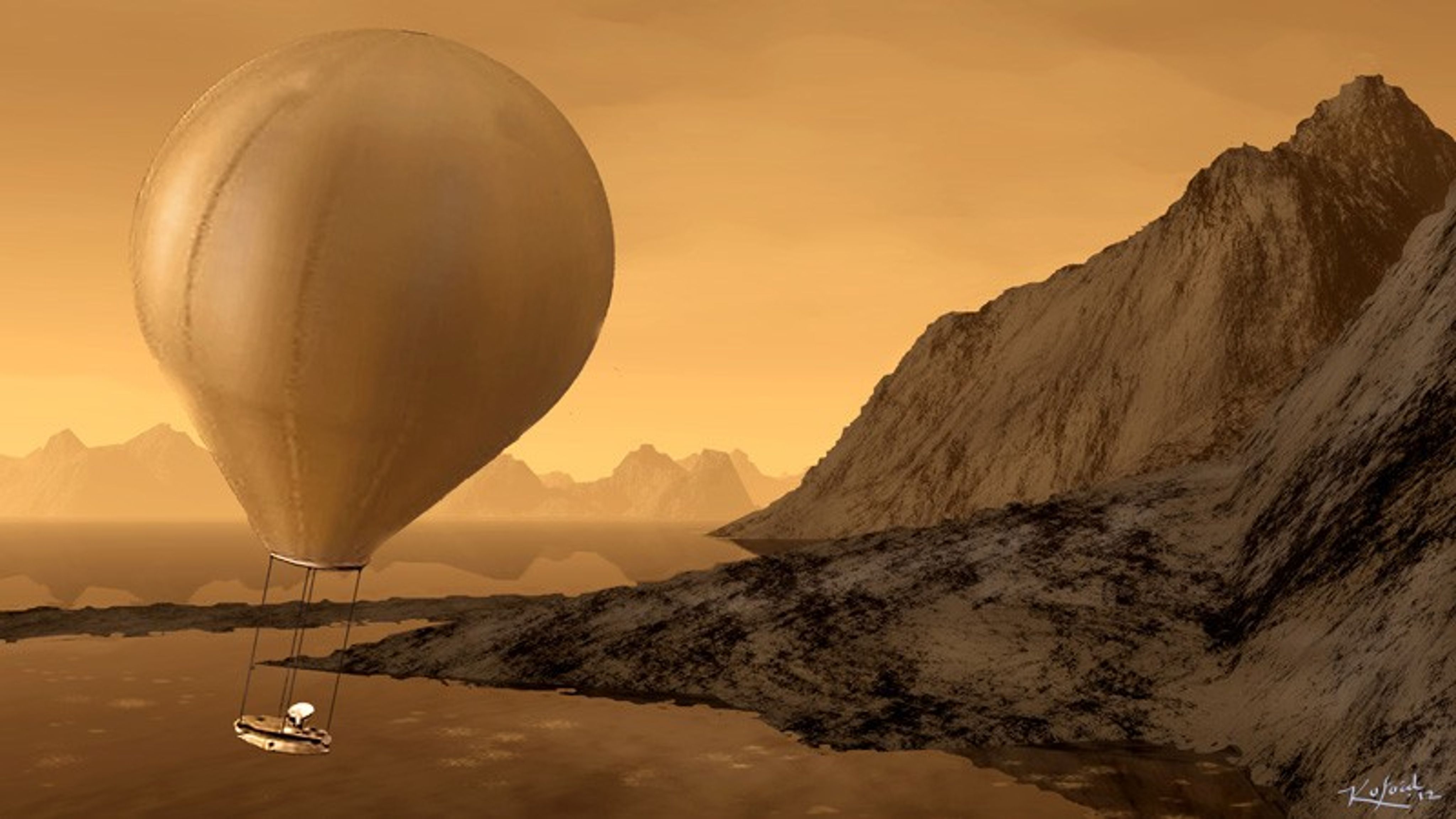
One of the major advantages of having a spacecraft land on the surface of a planet is that it can take direct measurements of the soil. The several Soviet Venera landers accomplished this on the 900° F surface of Venus, and the Viking landers accomplished this on the surface of Mars. Samples are taken from the soil and transported into the spacecraft's instruments where they are analyzed for chemical composition, and the data are relayed back to Earth.
This is all good, but the scientific community really would like to directly examine samples in the laboratory. A robotic sample return mission to Mars could be in the future. NASA has conducted long-range studies and technology development and may continue to do so for planning purposes. Several different scenarios are envisioned for accomplishing this, some of which would include a rover to go around and gather up rock and soil samples to deposit inside containers aboard the return vehicle. Interest in returning samples from Mars has heightened recently in the wake of discoveries including recently flowing springs, and ancient lake beds on Mars. Such samples would be examined for fossil evidence of life forms.
Sampling of cosmic dust in the vicinity of the Earth has also become an endeavor of great interest, since interplanetary dust particles can reveal some aspects of the history of solar system formation. Space shuttle experiments have so far been successful at capturing three 10 µm particles from Earth orbit, one intact.
Launched in 1999, Stardust collected samples of material from the coma of Comet Wild 2 in January 2004, for return to Earth January 15, 2006. The return capsule's entry, descent, and landing on Earth were flawless, and scientific analysis of its thousands of samples has yielded new insights about the composition and formation of comets. Genesis succeeded in returning samples of the solar wind, despite its unexpectedly hard landing on Earth in September 2004, when its parachute failed to deploy.
A spacecraft isn't always needed if you want to collect interplanetary material. Dust from interplanetary space rains continuously into Earth's atmosphere, which slows it gently because of the particles' low mass. In 1998 the NASA Dryden Flight Research Center flew one of its ER-2 high-altitude research aircraft with an experiment for the Johnson Space Center that collected high-altitude particulate matter -- or "cosmic dust" -- on two collector instruments mounted on pods under the wings.

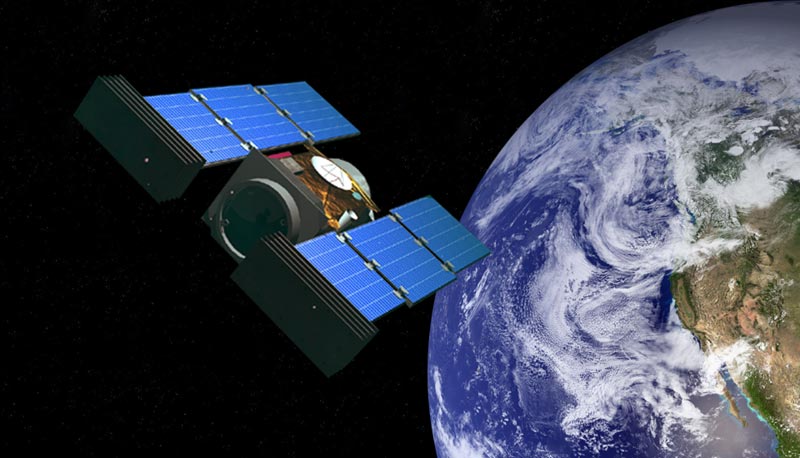